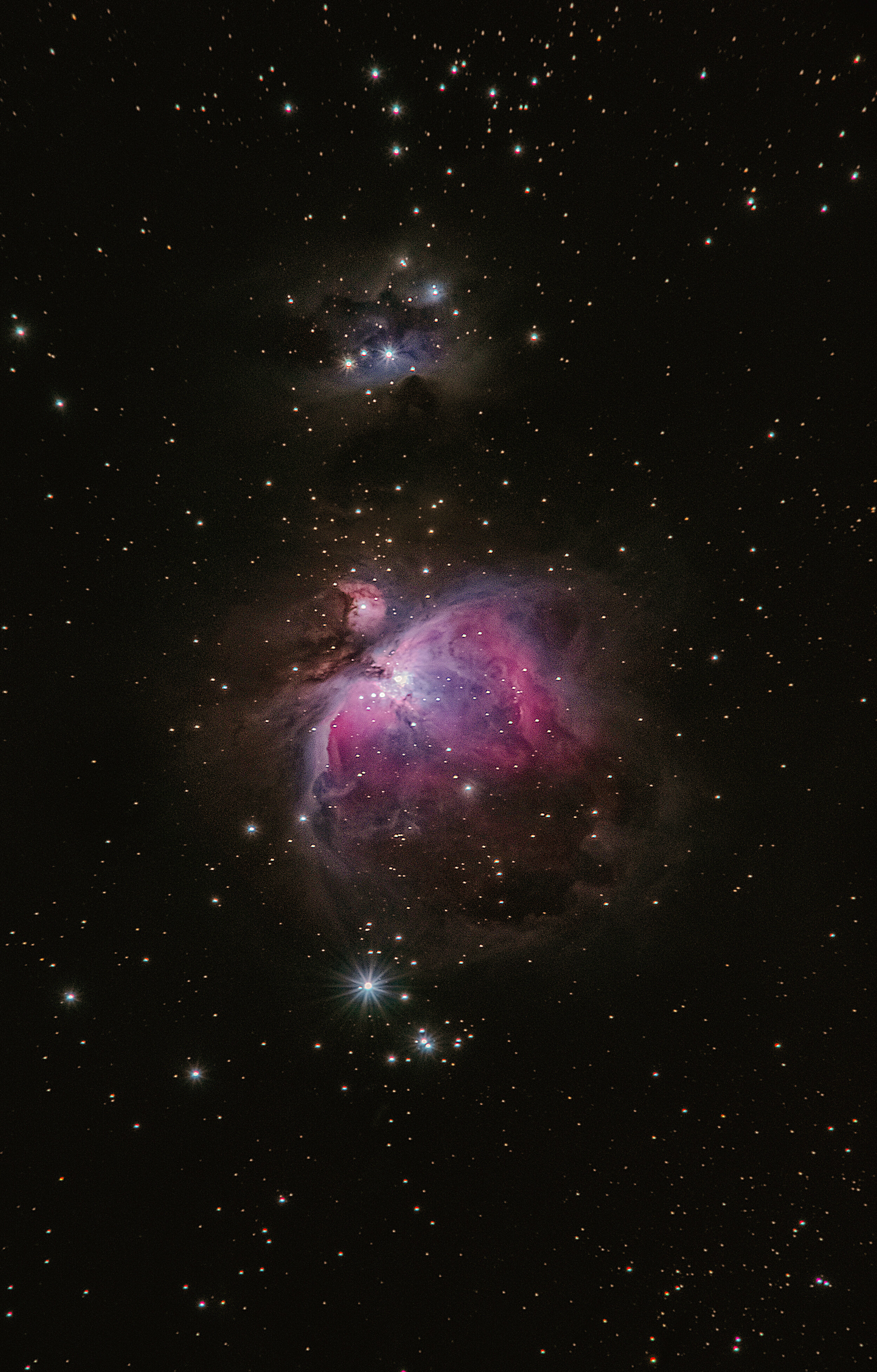
THE Closest supernova of the decade
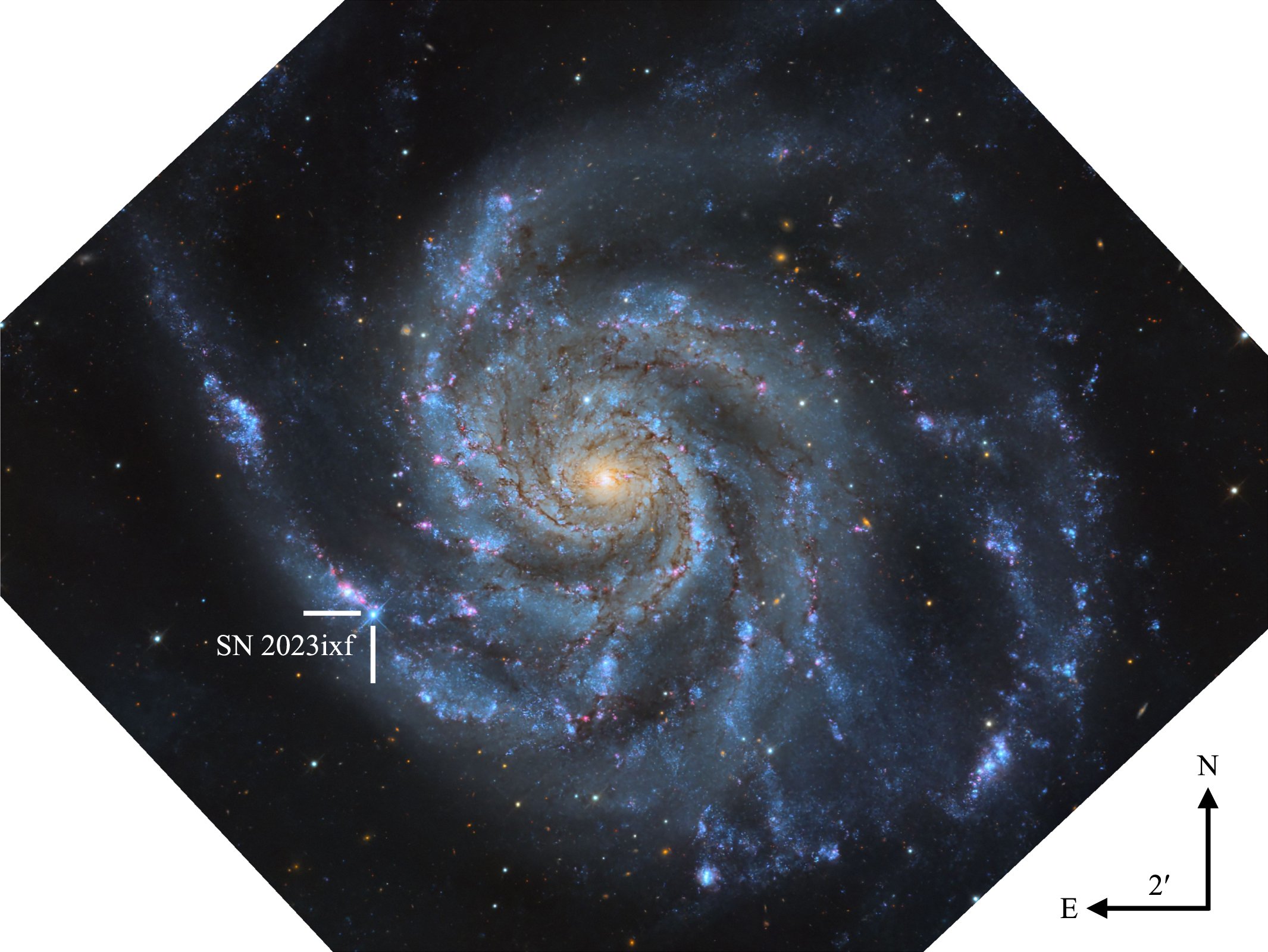
Supernova 2023ixf
On May 19 2023, amateur astronomer Kōichi Itagaki reported the discovery of a new supernova in the Pinwheel spiral galaxy (aka Messier 101). Soon named SN 2023ixf, this explosion represented the closest (and one of the brightest) supernovae in the last decade at a distance of only 6.9 Mpc. Early spectroscopic and photometric observations of SN 2023ixf revealed that it was belonged to a rare sub-type of core-collapse supernova from a red supergiant star that lost substantial mass right before explosion. Below you can find a summary of my paper on SN 2023ixf that utilized data from the Young Supernova Experiment (YSE) survey and Lick Observatory. This work was also highlighted in a press article in Scientific American.
Figure 1
After core-collapse, the expanding supernova shockwave bursts out of the red supergiant surface and collides with gas that the star had ejected in the final days to years before explosion. Observationally, this manifests as narrow emission lines of highly ionized gas in the supernova spectrum ~days after explosion.
Figure 2
The emission line dominated spectrum is a result of continuous ionization of the circumstellar gas by X-ray photons emitted at the supernova shock front. Prior to SN 2023ixf, our team already had model predictions (shown in red) of what the spectra would look like for such a process and it allowed us to constrain the density of the circumstellar environment as well as the mass-loss history of the red supergiant progenitor star prior to explosion. The best matched models suggest that SN 2023ixf arose from a red supergiant star that ejected ~0.04 - 0.07 solar masses worth of material in the last 3-6 years before collapsing.
Pre-explosion emission in red supergiants

Supernova 2020tlf
The final moments of a red supergiant’s life before supernova is almost completely unknown. However, a major breakthrough in our understanding of red supergiant evolution came from the discovery of luminous precursor (i.e., pre-explosion) emission prior to the type II supernova (SN) 2020tlf. This was the first time that we had observed pre-SN activity from a red supergiant that then exploded as a normal type II SN. Below is some selected press on this discovery as well as a summary of the paper on SN 2020tlf.
PRESS
Below is a summary of My paper on SN 2020tlf:
Figure 1
Marked in red is SN 2020tlf in its spiral host galaxy NGC 5731 as imaged by the Young Supernova Experiment transient survey.
Figure 2
SN 2020tlf is the first normal type II supernova to have a precursor detection prior to explosion. This emission was detected with the PS1 telescope as it imaged the explosion site for hundreds of days prior to the supernova. A direct image of the precursor emission is shown in the bottom panel after the template image (top) is subtracted from the science image (middle).
Figure 3
Using the pre- and post-explosion imaging of SN 2020tlf, we were able to construct a bolometric light curve sequence (shown in green) of both the progenitor star and the resulting supernova. Prior to explosion (t = 0), we saw luminous emission from the red supergiant progenitor star as it was gearing up for core-collapse.
Figure 4
The first spectrum taken of SN 2020tlf revealed that the explosion’s shockwave had collided with circumstellar gas that the progenitor had expelled right before explosion. Modeling of the narrow emission lines from produced by this process revealed that the progenitor experienced enhanced mass loss right before explosion.
Figure 5
Combining all the observations and modeling of SN 2020tlf and its precursor allowed us to create one of the most accurate pictures of a red supergiant’s final moments to date. Left is an animation showing the progenitor star expelling material before explosion; this gas being the cause of the narrow emission lines in the first spectrum and the luminous pre-SN emission.
Calcium-Rich Transients
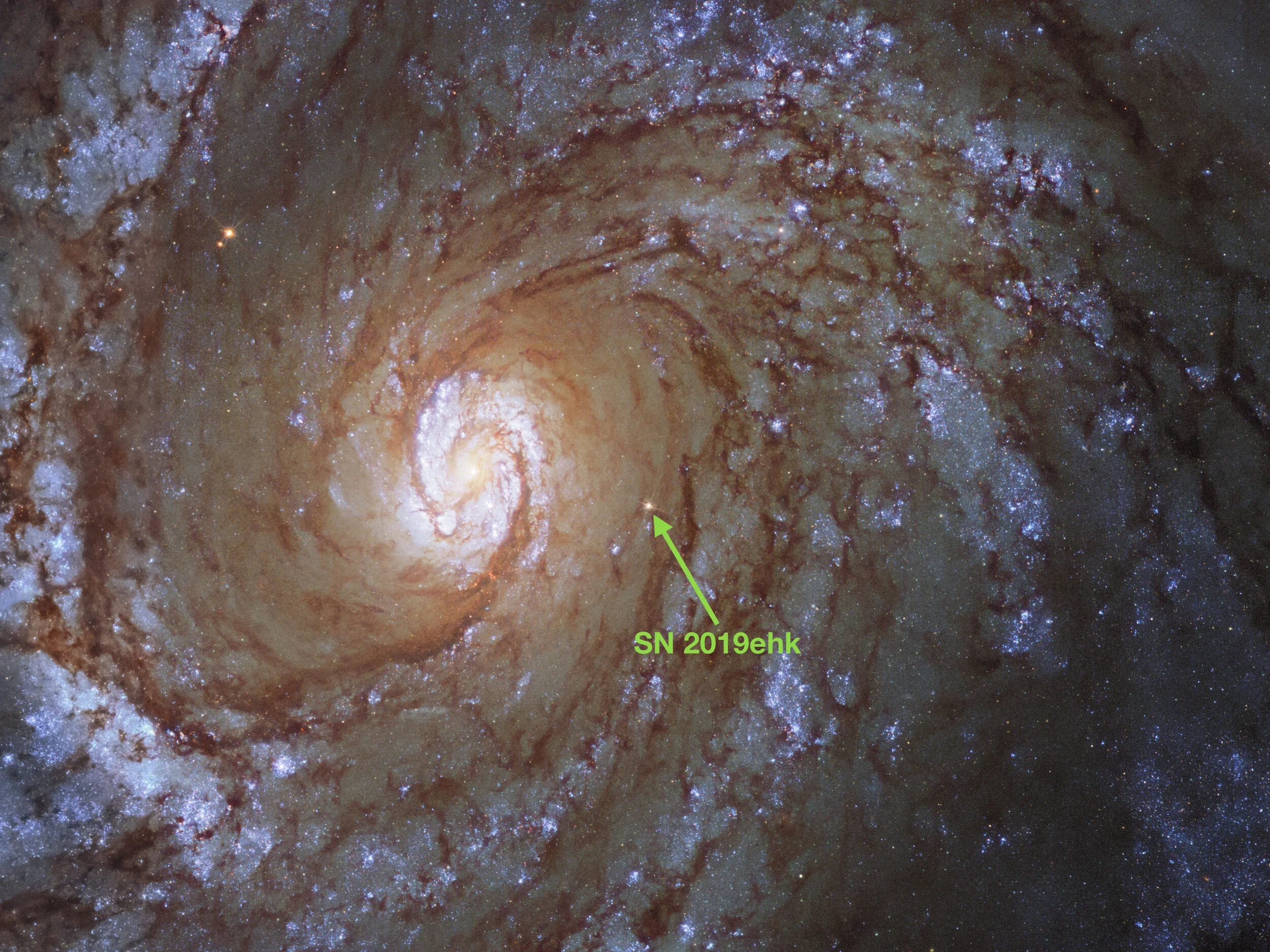
Supernova 2019ehk
Last year, a peculiar transient called SN 2019ehk was discovered within half a day of explosion in the nearby galaxy M100. This supernova (SN) belongs to the “Calcium-rich” transient class, which are distinguished by dominant Calcium emission in their spectra and a rapidly evolving, low luminosity light curve. These objects represent a relatively new class of explosions whose progenitors are completely unknown. Thanks to a global collaboration, we were able to study this SN with observations spanning the electromagnetic spectrum (x-ray to radio). This analysis placed the tightest constraints on the progenitors of this class to date.
Press:
Below is a summary of my recent paper on this intriguing object:
Figure 1
SN 2019ehk’s light curve has two peaks, which is unique for Ca-rich transients as well as SNe in general. This SN also emitted luminous X-rays that were coincident with the first peak, suggesting a linked emission mechanism. Theoretical predictions suggest that the first peak can arise from a SN shock colliding with extended material in the pre-explosion environment.
Figure 2
The name “Calcium-rich” is derived from an observation properties of SNe in this class. As shown to the right, the spectra of Ca-rich objects are dominated by Ca II and [Ca II] emission once the SN has become optically thin i.e. "nebular.” A SN is “Ca-rich” if the ratio of [Ca II] to [O I] emission is greater than 2. In SN 2019ehk, the ratio of [Ca II] to [O I] is over 30 making it the most “calcium-rich” SN ever discovered!
Figure 3
As soon as SN 2019ehk was discovered, a spectrum was obtained of the explosion (left). As shown to the left, this spectrum showed “flash-ionized” Hydrogen and Helium emission lines that form when the SN shock collides with gas in the progenitor environment. This observational signature had never been seen before in a Ca-rich SN and revealed that their progenitors eject mass into their local environment before exploding.
Figure 4
SN 2019ehk is the first Calcium-rich transient with pre-explosion imaging. We searched 2 decades worth of archival Hubble Space Telescope images but found no visible progenitor of the SN. As shown in the Hertzsprung-Russel diagram to the right, we can exclude all single, massive stars >8 Msun (pink region) as the progenitor of SN 2019ehk.
Figure 5
Left, a visual representation of SN 2019ehk derived from different explosion properties. From pre-explosion imaging, this system is either a massive star in a binary (9-10 Msun) or a white dwarf with a binary companion. The double-peaked light curve, coincident with X-ray emission and “flash-ionized” spectral lines, is strong evidence for circumstellar material (CSM, sea foam green) made of Hydrogen and Helium surrounding the progenitor star. When the star exploded, the SN shock interacted with this material, which caused these different observation signatures.
Helium Shell Detonations on White Dwarfs
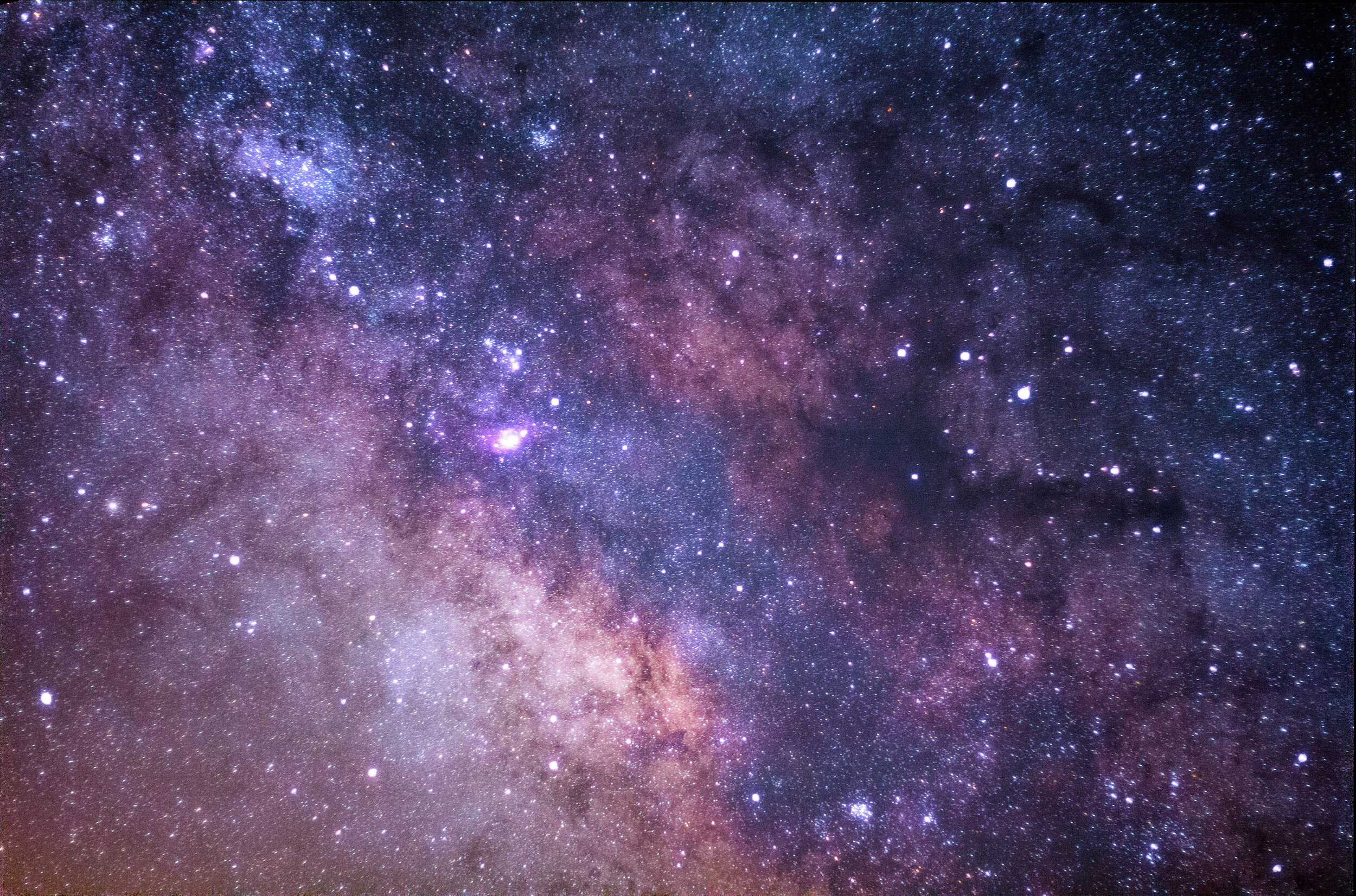
Supernova 2016hnk
Theoretical astrophysicists have no shortage of ways they can blow up white dwarfs (WDs). One such model is the helium shell double detonation: a WD accretes helium from a companion, which then detonates on the stellar surface and leads to a second detonation of the entire star. The resulting SN can take a variety of forms and these simulations have predicted what the explosions spectroscopic and photometric evolution should be, if such an event is discovered. Below I summarize a recent paper on the discovery of SN 2016hnk, which is best modeled by a helium shell detonation scenario.
Figure 1
SN 2016hnk is a peculiar thermonuclear supernova that shows many observational similarities to both Type Ia SNe and Calcium-rich transients. It is intrinsically red in color, has a low luminosity relative to other SNe Ia and its spectra are dominated by high velocity Calcium lines that continue out to late-times.
Figure 2
Left, a 2D visualization of a helium shell double detonation model by Polin et al. 2019a. Here the first shell detonation happens on the WD edge and then triggers a second detonation of the star’s core. This model will pollute the SN ejecta with heavy elements that will suppress the radiation coming from “bluer” wavelengths.
Figure 3
SN 2016hnk is observationally distinct from typical WD explosions e.g., SNe Ia. Consequently, we model its light curve (right) with the helium shell detonation of a WD below the Chandrasekhar mass limit. The model shown in green is the result of a 0.85 Msun WD that detonates with 0.02 Msun of helium on its surface. This model is consistent with other wavelengths outside of i-band photometry shown in orange.
Figure 4
Remarkably, this helium shell model in green also reproduces the observed spectral evolution of SN 2016hnk at early (left) and late-times (shown in paper). SN 2016hnk is now the third observed helium shell detonation of a WD and future sky surveys will most likely find many more for us to study!
TypE Iax Supernovae

Progenitors of SNE Iax
Thermonuclear supernovae come in all shapes and sizes. One peculiar sub-class of objects are Type Iax (SN Iax) supernovae: explosions that are have low kinetic energies, low ejecta velocities and lower peak luminosities than typical Type Ia supernovae (SNe Ia). Here I summarize a recent paper on the modeling of all known SNe Iax and the resulting constraints made on their unknown progenitors. (Press)
Figure 1
While their progenitors of these SNe are currently ambiguous, observational evidence indicates that they may be the result of a white dwarf (WD) accreting mass from a helium star companion. On the left is such a scenario wherein the WD grows in size as it gains mass from its companion Helium star. Once it reaches the Chandrasekhar limit (~1.4 Msun) the WD will explode and, under the right explosion physics, produce a SN Iax.
Figure 2
The explosion scenario described above is motivated by the detection of a blue, likely helium-rich, progenitor star in pre-explosion images of SN Iax, 2012Z (to the right). Furthermore, there have also been two other SNe Iax that show prominent helium emission in their spectra: SN 2004cs and SN 2007J.
Example of SYNAPPS spectral modeling of SNe Iax, 2014dt.
Top: Data in black, model in green.
Middle: Subtracting model from the data yields residuals. We then cross-correlate residuals with helium emission lines (red) to search for a detection.
Bottom: Results from cross-correlation show no evidence for positive detection of He I in SN 2014dt. Using this method, the combination of helium detections and non-detections allows us to understand statistically how many SNe Iax progenitor systems contain helium.
Figure 3
Motivated by the potential helium composition of the companion star, this paper focused on determining the presence of elemental helium in the spectra of SNe Iax and the relative amount needed for detection. To accomplish this, we modeled spectra of all known SNe Iax using spectral synthesis codes SYN++ and SYNAPPS (left). These spectral models fit a given spectra to determine the relative strengths of all ions present during the explosion. These models were run on the NERSC supercomputing facility at Lawrence Berkeley Labs (Press). This work places limits on the amount of helium in these systems and constrains the explosive mechanisms responsible for SNe Iax.
Typa Ia Supernovae

Progenitors of SNe Ia
We are now relatively certain that the thermonuclear explosion of a white dwarf (WD) will produce a Type Ia supernova (SN Ia). Astrophysicists are, however, divided on the identity of the WD’s binary companion: is it another WD or a larger, non-degenerate star? Below I describe a recent paper that utilized the rare collection of extremely late-time photometric imaging of Type Ia SN, 2013aa in order to probe the unknown progenitors of the class as a whole.
Figure 1
SN 2013aa is a nearby SN Ia in host galaxy NGC 5643. The SN is highlighted by red lines to the left in a false-color RGB image using the Swift space telescope.
Figure 2
Using Hubble Space Telescope (HST) images of SN 2013aa, we detected the source at over 1500 days after the explosion’s maximum light. In the right figure, a Gemini explosion image of SN 2013aa (top left panel) is compared to an HST image at ~1500 days since explosion (bottom left panel). Only performed on four other SNe Ia, this detection of SN 2013aa marks the second latest photometric detection of a SN Ia to date.
SN 2013aa at marked by red lines at early and late-times.
Figure 3
By examining the supernova’s luminosity decline with respect to time, we calculated the abundances of radioactive decay elements (Co-56, Co-57 and Fe-55), all of which power the explosion at very late-times. Models of the radioactive decay powered light curve shown to the left. Each fit is dependent on the physics of the explosion and the total masses of radioactive decay elements produced.
Figure 4
We then compared the relative masses of these elements to those predicted in different 3-D explosion models for SNe Ia, which tentatively indicated a consistency between SN 2013aa and a double white dwarf progenitor system. However, as shown in Figure 4, explosion models for SNe Ia are wide ranging; we are in need of better observations of nearby SNe Ia combined with detailed modeling that can predict explosion observables.
Explosion models compared with the estimated mass ratios (y-axis) and light curve stretch/broadness (x-axis) for all 5 SNe Ia observed at extremely late times.
Orbital Stability in ExoplaneTary Systems
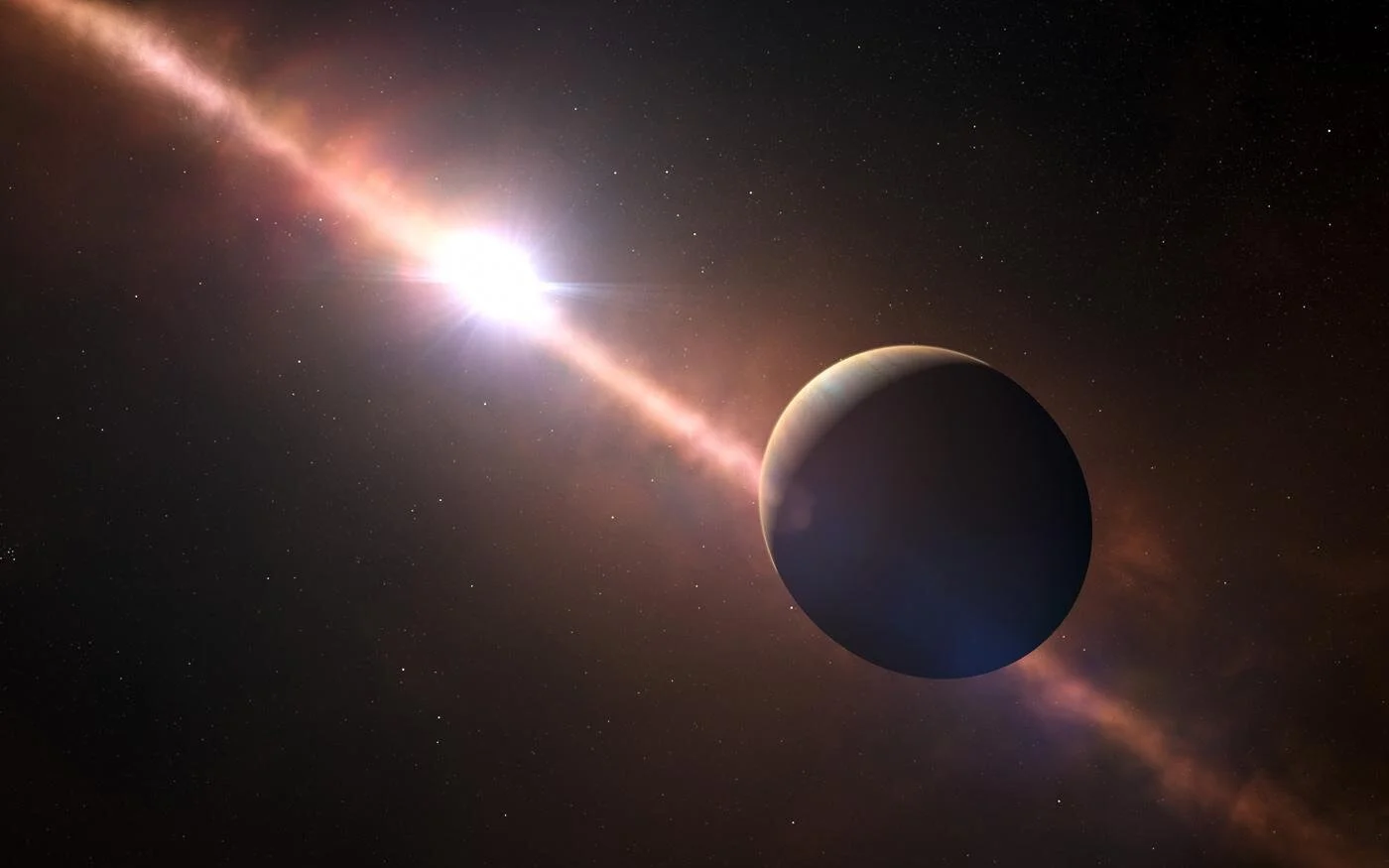
Stability in Exoplanets
The Kepler and TESS space telescopes have discovered thousands of planets orbiting distant stars. However, it is still very much a mystery (i) how these exoplanetary systems came to form and (ii) how do they remain in stable orbits for billions of years. Below I summarize a paper that I worked on that employed a massive parameter search to simulate stable orbits of observed exoplanetary systems and understand the physical properties of the planets involved.
Figure 1
Shown to the left is a visualization of the radial velocity (RV) method for detecting exoplanets. We modeled RV data of exoplanetary system HD 200964.
Figure 2
RV data from exoplanetary system HD 200964. Our models confirmed that this system consists of two Jupiter sized planets with orbital periods of ~600 and 800 days.
11 parameter MCMC model of two planets in HD 200964.
Figure 3
One of the most difficult problems with modeling exoplanetary orbits is keeping the planets on stable orbits for > billion years. We attempted to tackle this problem with a Markov Chain Monte Carlo grid search (left) to fit the RV data with physical parameters of the orbit e.g., planet masses, periods, eccentricities etc. We were able to recover long term stability for HD 200964 and discovered the ratio of orbital periods (resonance) is an essential element in simulating stable orbits.